Nanoenergetic Materials for Microscale Tactical Applications
James J. Valdes, PhD
Richard S. Potember, PhD
Diane M. Kotras
Download the PDF 
Military missions require small energy-dense formulations to power future generations of miniature autonomous systems and satellites, and to provide sufficient destructive energy yields in small explosive payloads. The weapons effects will need to be tailorable; that is, tuned for explosive yield or impact resistance, and the materials sufficiently robust to operate within the unique constraints of hypersonic missiles that are subject to extreme aerodynamic forces. In addition to their formulation as explosives and propellants, nanoenergetic materials can also be employed as pyrotechnics for breaching fortifications by destroying advanced materials such as ceramics, composites, and metal alloys, which are used for collective protection, and in antitamper devices and systems. In addition, their superior energy density relative to traditional mono-molecular materials such as TNT make them ideal candidates for future energy generation and storage devices. The figure illustrates the range of applications of nanoenergetic materials.
A key reason to explore nanoenergetic materials for fuels and propellants hinges on potential improvements resulting from altered chemical kinetics rather than thermodynamics. For example, 1-nm aluminum particles (nanoscale) reacting with oxygen release only 1.04 times as much energy as does ultrafine aluminum (traditional micron particle-size range), but the rate of energy release (i.e., the kinetics) of the former is potentially faster because the balance of the rate-controlling factors shifts as the particle size is reduced.1 This is because the rate of combustion, or the balance of the generally slower mass transport rates and faster chemical reaction rates, controls the explosion process.
By contrast, the opposite is true of nanomaterials: the high surface area of small particles and the short diffusion length between particles are expected to enhance the role of chemical kinetics, an important consideration in designing energetic materials because particles that are closer together are going to react more quickly.
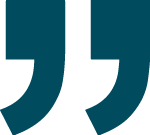
This fact frequently makes mass transport a controlling energy release process in conventional munitions and propellants in which micron and larger particle sizes of energetic materials are used. Mass transport simply means that with larger-scale traditional explosives, the longer distance between a molecule of fuel and a molecule of oxidizer is a more important factor than the speed or kinetics of reaction. By contrast, the opposite is true of nanomaterials: the high surface area of small particles and the short diffusion length between particles are expected to enhance the role of chemical kinetics, an important consideration in designing energetic materials because particles that are closer together are going to react more quickly. In addition, an unprecedented degree of control of the energy release rate may be possible by varying the composition on the nanodimensional scale. The burning rate might be accelerated, the delivered specific impulse, or shock wave, could be increased by improved combustion efficiency, and the detonation might achieve greater results while not increasing the size of the fuel package.2
Nanoenergetic materials such as nanothermites are generally formulated as an elemental metal such as aluminum combined with a metal oxide (i.e., a metal with an oxygen bond; for example, rust); the former is the fuel and the latter is the oxidizer.3 These have superior reaction rates and energy yields relative to their “meso-scale” traditional formulations and to conventional explosives but pose problems unique to reactions at these small scales. Recent advances in understanding the physical and chemical properties of nanomaterials have begun to address these problems, and formulations with superior energy yields now show promise for applications in miniature military systems and to be the next generation of explosives and propellants. This is due to their decreased sensitivity to impact, friction, and shock waves, and increased energy release and burning rate.4 These characteristics make them much safer to handle than current munition fills.
In addition to traditional military mission applications, there is an increased emphasis on the use of autonomous systems such as unmanned aerial, ground, and underwater vehicles, and other autonomous systems to perform surveillance, reconnaissance, search and recovery, and search and destroy missions. There are advantages to miniaturizing these systems such as signature reduction, ability to penetrate small, enclosed spaces, and reduced logistics. In addition, the potential for such microrobotic systems to be deployed as distributed, coordinated networks or swarms both increases mission flexibility and complicates an adversary’s countermeasures.
Space assets are now at risk from numerous potential adversaries, especially at the onset of a conflict, and will have to be rapidly reconstituted, most likely with swarms of “nanosatellites,” each about the size of a shoebox. Compact, energy-dense nanoenergetic materials will power the tiny thrusters needed to maneuver such satellites in orbit.
A nanometer is a unit of length in the International System of Units equal to one billionth of a meter (0.000.000.001 m). One nanometer can be expressed in scientific notation as 1 x 10 -9 or 1/1,000,000,000 meters. This article is focused on discussing the potential for generating greatly increased amounts of energy from the energy conducive properties of collected quantities of aluminum particulates (about one nanometer each in size) than can be generated by current materials. These would take less space, be lighter and more resilient to stress, and produce much greater amounts of energy for various uses than those currently employed.
Expected Operational Developments by 2040
The next twenty years will see the development of small, highly energetic explosive payloads for microdrones, which could be deployed in virtually undetectable swarms to disable power grids and communications networks, penetrate life support systems of underground facilities, and disable electronics and life support systems. In addition, smaller payloads for hypersonic missiles and conventional artillery will result in reduced logistics and smaller radar, heat, and optical signatures. Ultimately, the energetic yields of such materials could be dialed up or down to restrict collateral damage or maximize destructive effects.
The military will also need propulsion systems to enable maneuvering in orbit of critical space assets for communication and targeting.5 Nanoenergetic materials will be critical for solid fuel propulsion systems with much lighter weight and greater energetic yields than current solid fuel systems with minimal environmental impact.
Breaching and antimateriel applications rely on much the same chemistries as propellants and explosives but have their own unique requirements. These include stable and low-temperature ignition but extremely high-temperature combustion for breaching fortifications such as underground bunkers or destroying advanced materials such as ceramic composites and metal alloys, as well as for welding during forward repair or industrial manufacturing.
Finally, energy storage and power generation in forward-deployed units with limited logistics reach-back will require new energy dense materials for batteries with greater yields per mass than current materials.6
General Technology Limits
Controlling particle size of nanomaterials and their tendency to stick together in larger clusters during synthesis is a major challenge that has yet to be resolved. The goal is to prepare monodisperse particles—that is, particles of identical size and shape, in the optimal range of 10–100 nm, since metal particles smaller than 10 nm tend to be pyrophoric and spontaneously combust in the presence of an oxidizer such as air. New surface particle coating materials are needed to enhance energy content, control ignition temperatures, tailor the rate of combustion, and mitigate processing issues.7
Ignition behavior of nanoparticles is not well understood, and most research has focused on aluminum. Therefore, a systematic analysis of other elements and chemical compounds that may be superior to aluminum is needed. A better understanding of the thermomechanical properties of the oxide layer, a layer that spontaneously forms and interferes with combustion, on nanoparticles is required to develop a complete model for particle ignition. Combustion temperatures are dependent on several properties, and the relative importance of vapor-phase and surface reactions is not currently sufficiently worked out to allow for the predictive modeling needed to tailor the materials.8
Nanofluid fuels hold great promise, but some basic mechanisms need to be examined. These include the relative catalytic and thermal effects of the addition of nanoparticles to fuel, the agglomeration of the nanoparticles in the fuel that can lead to uneven burn, and the multiphase flow dynamics in the exhaust nozzle of rocket engines. In short, will adding nanoparticles enhance propellant burn or have unpredictable negative effects on the combustion process?
This article is organized according to the three operational capabilities, which the authors posit as the prime beneficiaries of advances in nanoenergetic materials. The capabilities are described generally in terms of current performance and technological advances required within the year 2040 time frame, which was selected to align with Under Secretary of Defense for Research and Engineering mission-level assessment exercises. These operational capabilities are explosives for improved weapons effects; propellants for terrestrial, underwater, and space systems; and breaching and antimateriel operations.
Explosives for Improved Weapons Effects
General background. We have reached the limits of energy that can be released as an explosive or pyrotechnic using traditional chemical formulations, which rely on the energy inherent in CHNO—carbon, hydrogen, nitrogen, oxygen—chemical bonds.9 Weapon systems benefit by reducing their size, hence signature and logistics burden, and by the ability to tailor weapons effects depending on mission requirements. New energetic materials with increased yield and more flexible applications are required.
Current explosives such as HMX, RDX, and TNT are monomolecular formulations in which fuel and oxidizer groups are present on a single molecule and the rate of reaction is determined by breaking chemical bonds. The speed of the reaction—hence the explosive power—is largely determined by mass-transfer limitations; that is, the larger the particles, the slower the speed of reaction, and the density of these formulations is very limited.10
Adding metal particles, particularly aluminum, to pyrotechnics, explosives, and propellants is known to increase the energetic output.11 Future explosives will incorporate metallic and other energetic nanoparticles and a nanoparticulate oxidizer to greatly increase the surface areas for reaction, thus liberating vastly more energy in a shorter time than conventional explosives. They will also be tunable for specific weapons effects by manipulating the chemistries of the surfaces of the particles, or by introducing fluidizer components.12
Technology challenges. The combination of molecular self-assembly techniques, colloquially referred to as “crock-pot chemistry,” and supramolecular chemistry, a field that studies the bonds between different molecules rather than within a single molecule, will result in next generation nanoenergetic materials. For example, self‐assembled nanocomposites significantly improve combustion performance in aluminum and bismuth trioxide nanoparticles.13 Graphene oxide directed self‐assembly can be used to synthesize nanocomposites with diverse combustion properties and controlled ignition sensitivity and directed self‐assembly lays the foundation for preparing multifunctional, highly reactive combustion systems in the future. Metal nanoparticles smaller than 10 nm are pyrophoric, meaning they can ignite spontaneously in the presence of air, which poses a significant safety problem. The challenge is to produce uniform particles at sizes within the critical range of 10–100 nm.
The percentage of atoms on the surface of a particle increases from 2 percent to 92 percent as the particle size decreases from 100 nm to 1 nm, and the surface atoms are much more energetic that those in the particle core.14 This increases speed and completeness of reactions. Conversely, formation of an oxidation layer on the surface, which can represent 60 percent of the mass of the particle, will have analogous outsized effects and, depending on the material, could increase or decrease energy content and shelf life. The ideal energetic material is composed of uniformly sized and dispersed unoxidized metal clusters. Clusters of metals have been shown to mimic the properties of one or more elements and have been called “super atoms.” This suggests the use of clusters as basic building blocks for new classes of nanoscale materials with tailored properties.15 The technical challenge is to develop techniques for the directed design of metal clusters with specific particle and cluster sizes and distribution and relate that to physico-chemical characteristics. That is, how do size, shape, and clustering of particles affect their properties as explosives or propellants? Current manufacturing methods have some drawbacks, including incomplete heat transfer to precursor molecules, deposition of clusters on the furnace wall, and impurities from reactor walls. These issues can be minimized by using combustion flame chemical vapor condensation (CF-CVC) methods.16
Ignition of metals is caused by phase transformations—rapidly transitioning from a solid to a gas—of the metal core and/or the oxide layer and tend to occur at high temperatures. The ideal material would have a low ignition temperature and ignition delays, which would maximize energy release rates. Combustion can take place in the vapor (gas) phase away from the metal core, at the particle’s surface, or both. A complete model of particle ignition and combustion has not been developed and such a model would enable one to tailor different materials for specific energetic effects.
Supercomputing platforms are facilitating first-principles-based simulations to predict behavior of complex systems never previously achieved.17 These simulations can integrate chemical reactions at the atomic level and mechanical processes at the meso-scale to solve mechano-chemistry (i.e., chemical reactions under mechanical stress) problems such as the sensitivity of high-energy density nanomaterials whose chemical reactions undergo shock waves.18 Within a decade, supercomputing performance has progressed from 0.478 petaflops (1015 floating-point operations per second, or one thousand million million operations) for solving linear systems of equations to 148.6 petaflops in the summit supercomputing machine at the Department of Energy’s Oak Ridge National Laboratory.19
The Collaboratory for Advanced Computing and Simulations at the University of Southern California successfully tested simulation frameworks on multiple parallel supercomputers as well as on a grid of six supercomputers in the United States and Japan to assess the scalability of molecular dynamics and quantum mechanical algorithms. They also used an additional embedded cellular decomposition simulation framework to determine how processes at the atomic level inform material processes such as nanoindentation on nanocomposite materials, oxidation of nanoenergetic materials, hypervelocity impact damage, fracture, and the interaction of voids with nanoductility.20 They used ab initio molecular dynamics simulation (simply put, simulation of complex molecular systems and processes on a computer) to evaluate the various electronic processes that occur during a thermite reaction. The quantum mechanical simulation further allowed a quantitative study of the combustion rates that could not be explained by conventional diffusion-based mechanisms.21 Their collective work resulted in the development of high-end reactive simulation programs at the atomic level, which are critical for developing new nanoenergetic materials by enabling billion-atom simulations of mechano-chemical processes.22
Tunable Propellants for Terrestrial, Underwater, and Space Systems
General background. Solid-state propellants have several operational advantages relative to liquid fuels such as ease and safety of handling and superior readiness to launch. However, they are limited in their energetic yields and ability to control burn rates. The space shuttle’s solid-state boosters used a composite energetic material consisting of ammonium perchlorate crystals and aluminum particles in a polymer binder to increase energy yield and burn rates. This is a well-known approach to increasing the energetic yield of solid propellants used in strategic ballistic and tactical missiles. Future hypersonic missile systems will require new, more powerful solid fuel compositions while providing improved shelf life, stability, and safety over current solid fuel systems.
Future formulations incorporating energetic nanoparticles will have superior energy density relative to conventional solid fuels, making them suitable as propellants for both missiles and small space assets. They will be tailorable for burn rates and will have increased production, storage, and safety handling characteristics as well as reduced human and environmental toxicity.23 In addition, underwater propulsion could use aluminum-water reactions to power unmanned underwater vehicles.24
Hypersonic missile technology is a priority for Russia, China, and the United States. Some systems use liquid fuels, but others will be developed using solid fuels, which are advantageous because they do not require fueling just prior to launch. Current solid fuels under investigation are now limiting high Specific Impulse (Isp) characteristics to below 273 since high Isp fuels have been banned from U.S. Navy ships and submarines due to the explosive hazards they present. As fuels for hypersonic missiles mature, a greater emphasis will be placed on using insensitive munitions (IM), which are stable enough to withstand shocks, vibration, fire, and impact by shrapnel but can explode on target as intended.
Technology challenges. The technical challenge in manufacturing is spatial patterning to assure optimal proximities of oxidizer and fuel molecules. The closer and more evenly spaced the molecules are, the more efficient the reaction. The enabling technologies are polymer chemistry, micro-emulsion synthesis, cage and cluster chemistry and, more recently, artificial intelligence (AI)-driven modeling and simulation. The great contribution of AI is the ability to review vast quantities of data and identify obscure relationships and patterns which elude the human brain. The nexus between AI and modeling and simulation (M&S) is worthy of exploration in the context of examining its potential to inform computer simulations focused on both the reactivity and decomposition of nanostructured materials. While much work has been done on simulations of nanoenergetic particles, the extrapolation into the realm of AI M&S and its potential for designing new molecules remain relatively unrealized.25 Previous research on the intersection of AI and M&S underscores how the latter, with its inherent problems and challenges, may benefit from AI concepts and techniques.26 Simulation has been used to develop AI applications including how AI components can be inserted into a simulation to establish machine learning or adaptive behaviors, key aspects in determining structure-function relationships at the molecular level. Simulation is now recognized as having the ability to evaluate the impact of incorporating AI into real world systems such as manufacturing processes.27 Another innovative opportunity in M&S is the application of AI for production process optimization and calibration.28
The application of AI M&S to nanoenergetic particle research creates multiple opportunities. Advances in AI M&S can lead to future identification and development of nanoenergetic materials, including new methodologies for assembling these materials in specific functional architectures (say, propellants vs. explosives) with concomitant increases in performance and managed energy release rates. These advances can also inform new and more innovative applications of their use.29
Breaching and Antimateriel
General background. Advances in ceramics, carbon fiber composites, high density concrete, and metal alloys will create new advanced materials which are resistant or impervious to current munitions. Special operations, whether terrestrial or underwater, will require new super-energetic materials which are stable and have low ignition temperatures but extremely high combustion temperatures. Nanothermite metastable intermolecular composites (MICs), discussed in a previous section, can be formulated into thin films, metal organic frameworks, nanothermite colloids, and other formulations which will have these desirable characteristics.30
Current nitramine explosives such as RDX and HMX and formulations such as Semtex (RDX and PETN) are used for demolition, and recent experimental formulations using nano-nitramines show a roughly 60 percent decrease in shock sensitivities, hence a significantly increased safety factor. Nanothermites are the only nanoenergetics currently used in the military.31
Nanoenergetics will move beyond aluminum-based nanothermites with resulting decreases in sensitivity, increases in energetic yields, and capacity to tune these characteristics to mission requirements. Novel formulations such as tapes and foils for use in special operations will also be available. Production techniques such as inkjet printing with > 90 percent nanoenergetic materials and < 10 percent polymers or microsphere technology will enable development of stable but highly energetic strips or sticks.32
Technology challenges. Going beyond aluminum-based nanothermites to other metals as fuels, to surface functionalized or energetically filled carbon nanotubes, or to new cluster chemistries will require a “rules-based” approach to molecular screening, and practical applications will be facilitated by ordered molecular self-assembly. AI algorithms for determining structure-function relationships, discussed in an earlier section of this article, will also be critical.
New energetic materials must have low sensitivity to shock, low pyrophoricity, low ignition temperatures, and high combustion rates to be viable candidates to replace current propellants and explosives. Ignition and combustion physics are not currently well understood in a comprehensive manner and a predictive model is required as materials go beyond aluminum-based nanothermites into more exotic chemistries. The technical challenge is to develop optimal ignition and combustion characteristics to enable the energetic material to breach a diverse set of metals, reinforced glass, and advanced polymeric materials used for personal and collective protection.
As with all applications of nanoenergetic materials, the rate of ignition and combustion is affected by the oxide layer in the nanometer range that forms on the surface of the core particle. These layers significantly reduce the energy content of the particles at nanoscales.33 As previously mentioned, coatings such as boron can enhance energy release, while others can inhibit the reaction, a critical issue that all formulations must account for. The consummate coating material will amplify both energy content and shelf-life and facilitate ignition without impacting the low sensitivity characteristics.34 The technical challenge is selecting the ideal coating material and applying it in a uniform manner to enhance the energy content, improve reactivity, and reduce particle agglomeration.35
Conclusions
Future operational impact. Next generation space, terrestrial, and marine weapon platforms will require higher energy densities for explosive payloads, propellants for hypersonic missiles and nanosatellite thrusters, breaching materials for advanced ceramics and alloys, and lightweight energy storage. Any mission that requires the force to “shoot, scoot, or toot” while operating at a long distance from resupply will have significant logistical advantages in lighter weight and physically smaller packaging, increased lethality due to the advantages conferred by nanoenergetic materials on payload yields, and decreased time-to-target enabled by advanced propellants. In addition, the impact and friction resistance of these materials and their low ignition but high burn temperatures represent important safety advantages to both operators handling energetic materials and in manufacturing processes.
Future research directions. Thermite compounds have been studied extensively and there exists significant scientific literature in the field.36 More recently, an understanding of the techniques needed to produce uniform monodisperse nanoparticles and to passivate (coat) the surfaces to reduce oxidation have yielded an order of magnitude increase in reaction rates and energetic yields but may have reached the limits of these improvements. The recent design of new classes of highly energetic cage and cluster molecules—which increase energy density and solve the “architecture” problem of arranging the proximity of fuel and oxidizer—and the potential to organize these structures systematically in a manner analogous to the periodic table of elements appear to be the next advances in the design of nonthermite energetic materials with even greater reaction rates and energetic yields. As with any new material and especially those formulated at the nanoscale, the impact on both the environment and human health will need to be assessed using advanced toxicogenomic and toxicoproteomic assays that assess the potential toxic effects on human genes and proteins, respectively, as well as studies of the environmental microbiome, the beneficial microbes that inhabit the environment. Large-scale incorporation of these materials into weapon systems will also require the development of future manufacturing capabilities.
The authors thank Mrs. Donna M. Hoffman for her superlative review, recommendations, and preparation of the manuscript. This work was supported by MITRE’s IR&D Program, approved for Public Release, Distributed Unlimited, Case #22-3148. The figure was generated by and is the property of the authors.
Notes
- R. S. Averback et al., “Kinetic and Thermodynamic Properties of Nanocrystalline Materials,” Materials Science Society Symposium Proceedings (1989): 153.
- National Research Council of the National Academies, Advanced Energetic Materials (Washington, DC: National Academies Press, 2004), https://doi.org/10.17226/10918.
- Soo Hyung Kim and Michael R. Zachariah, “Enhancing the Rate of Energy Release from Nanoenergetic Materials by Electrostatically-Enhanced Assembly,” Advanced Materials 16, no. 20 (2004): 1821–26, accessed 13 December 2022, https://onlinelibrary.wiley.com/doi/abs/10.1002/adma.200306436.
- M. K. Berner, V. E. Zarko, and M. B. Talawar, “Nanoparticles of Energetic Materials: Synthesis and Properties,” Combustion, Explosion, and Shock Waves 49, no. 6 (2013): 625–47, accessed 13 December 2022, https://link.springer.com/article/10.1134/S0010508213060014.
- F. J. Diez et al., “On the Capabilities of Nano-Electrokinetic Thrusters for Space Propulsion,” Acta Astronautica 83 (2013): 97–107, accessed 13 December 2022, https://www.sciencedirect.com/science/article/abs/pii/S0094576512003773; Ivan Puchades et al., “MEMS and 3-D Printing Microthrusters Technology Integrated with Hydroxide-Based Nanoenergetic Propellants,” 2017 IEEE 37th International Conference on Electronics and Nanotechnology (ELNANO) (2017): 67–70, accessed 13 December 2022, https://ieeexplore.ieee.org/document/7939717.
- Terrence L. Connell Jr. et al., “Combustion of Alane and Aluminum with Water for Hydrogen and Thermal Energy Generation,” Proceedings of Combustion Institute 33, no. 2 (2011): 1957–65, accessed 13 December 2022, https://www.sciencedirect.com/science/article/abs/pii/S154074891000372X.
- Prawal P. K. Agarwal et al., “Surface Functionalized Boron Nanoparticles with Reduced Oxide Content by Nonthermal Plasma Processing for Nanoenergetic Applications,” Applied Materials and Interfaces 13, no. 5 (2021): 6844–53, accessed 13 December 2022, https://pubs.acs.org/doi/10.1021/acsami.0c20825.
- Dilip Srinivas Sundaram, Puneesh Puri, and Vigor Yang, “A General Theory of Ignition and Combustion of Nano- and Micron-Sized Aluminum Particles,” Combustion and Flame 169 (2016): 94–109, accessed 13 December 2022, https://www.sciencedirect.com/science/article/abs/pii/S0010218016300529.
- Michael R. Zachariah, “NanoEnergetics: Hype, Reality and Future,” Propellants, Explosives and Pyrotechnics 38, no. 1 (2013): 7, https://doi.org/10.1002/prep.201380131.
- T. M. Tillotson et al., “Nanostructured Energetic Materials Using Sol-Gel Methodologies,” Journal of Non-Crystalline Solids 285, no. 1-3 (2001): 338–45, accessed 13 December 2022, https://www.sciencedirect.com/science/article/abs/pii/S002230930100477X; A. E. Gash et al., “Nanoparticles,” in Energetic Materials: Particle Processing and Characterization, ed. Ulrich Teipel (Hoboken, NJ: Wiley, 2005), 238.
- Ying Zhu et al., “In Situ Preparation of Explosive Embedded CuO/Al/CL20 Nanoenergetic Composite with Enhanced Reactivity,” Chemical Engineering Journal 354 (2018): 885–95, accessed 13 December 2022, https://www.sciencedirect.com/science/article/abs/pii/S1385894718315377.
- Ho Sung Kim et al., “Tuning the Ignition and Combustion Properties of Nanoenergetic Materials by Incorporating with Carbon Black Nanoparticles,” Combustion and Flame 194 (2018): 264–70, accessed 13 December 2022, https://www.sciencedirect.com/science/article/abs/pii/S0010218018302001.
- Ling Chen et al., “Safe Fabrication and Characterization of NC/CL-20/CnMs Nanoenergetic Composite Materials via Modified Sol-Gel,” ChemistrySelect 5, no. 48 (2020): 15121–29, accessed 13 December 2022, https://chemistry-europe.onlinelibrary.wiley.com/doi/abs/10.1002/slct.202004386; Ling Chen, Weidong He, and Jie Liu, “Safe Fabrication, Thermal Decomposition Kinetics, and Mechanism of Nanoenergetic Composite NBC/CL-20,” ACS Omega 5, no. 48 (2020): 31407–16, accessed 13 December 2022, https://pubs.acs.org/doi/10.1021/acsomega.0c04958.
- Sundaram, Puri, and Yang, “A General Theory of Ignition and Combustion of Nano- and Micron-Sized Aluminum Particles.”
- A. W. Castleman Jr. and S. N. Khanna, “Clusters, Superatoms, and Building Blocks of New Materials,” Journal of Physical Chemistry 113, no. 7 (2009): 2664–75, accessed 13 December 2022, https://pubs.acs.org/doi/10.1021/jp806850h.
- Y. Chen et al., “High Rate Synthesis of Nanophase Materials,” Nanostructured Materials 9, no. 1-8 (1997): 101–4, accessed 13 December 2022, https://www.sciencedirect.com/science/article/abs/pii/S0965977397000287.
- Stephen Emmott, “Towards 2020 Science,” Science and Parliament 65, no. 4 (2008): 31–33.
- F. Shimojo et al., “Metascalable Molecular Dynamics Simulation of Nano-Mechano-Chemistry,” Journal of Physics: Condensed Matter 20 (2008), http://dx.doi.org/10.1088/0953-8984/20/29/294204.
- TOP500 Team, “TOP500 Becomes a Petaflop Club for Supercomputers,” Top500, 16 June 2019, accessed 13 December 2022, https://www.top500.org/news/top500-becomes-a-petaflop-club-for-supercomputers/.
- Shimojo et al., “Metascalable Molecular Dynamics Simulation of Nano-Mechano-Chemistry.”
- Ibid.
- Ibid.
- Luigi T. DeLuca, “Overview of Al-Based Nanoenergetic Ingredients for Solid Rocket Propulsion,” Defence Technology 14, no. 5 (2018): 357–65, accessed 13 December 2022, https://www.sciencedirect.com/science/article/pii/S221491471830045X.
- J. P. Foote, B. R. Thompson, and J. T. Lineberry, “Combustion of Aluminum with Steam for Underwater Propulsion,” in Advances in Chemical Propulsion, ed. Gabriel D. Roy (Boca Raton, FL: CRC Press, 2002), 1–16.
- Shimojo et al., “Metascalable Molecular Dynamics Simulation of Nano-Chemistry.”
- Bernard Ziegler, Alexandre Muzy, and Levent Yilmaz, “Artificial Intelligence in Modeling and Simulation,” Encyclopedia of Complexity and Systems Science (New York: Springer, 2009), 1–44.
- Lyle Wallis and Mark Paich, “Integrating Artificial Intelligence with AnyLogic Simulation” (presentation, 2017 Winter Simulation Conference, Las Vegas, NV, 4 December 2017).
- Ibid.
- Richard A. Yetter, “Nano Engineered Energetic Materials MURI” (presentation, Workshop on NanoEnergetics, Rutgers University, Piscataway, NJ, 28 February 2018), accessed 16 December 2022, http://coewww.rutgers.edu/~sdytse/NanoE-Workshop2008/Yetter.pdf.
- Zhang Wenchao et al., “Significantly Enhanced Energy Output from 3-D Ordered Macroporous Structured Fe2O3/Al Nanothermite Film,” Applied Materials and Interfaces 5, no. 2 (2013): 239–42, https://doi.org/10.1021/am302815y; Hatem M. Titi et al., “Metal-Organic Frameworks as Fuels for Advanced Applications: Evaluating and Modifying the Combustion Energy of Popular MOFs,” Chemistry of Materials 31, no. 13 (2019): 4882–8, accessed 13 December 2022, https://pubs.acs.org/doi/10.1021/acs.chemmater.9b01488; M. Gaber Zaky et al., “Nanothermite Colloids: A New Prospective for Enhanced Performance,” Defence Technology 15, no. 3 (2018): 319–25, accessed 13 December 2022, https://www.sciencedirect.com/science/article/pii/S2214914718303040.
- N. N. Perreault, “Potential Environmental Impact of Nanoenergetics,” European Chemical Bulletin 7, no. 3 (2018): 106–14, http://dx.doi.org/10.17628/ecb.2018.7.106-114.
- Haiyang Wang et al., “Direct Writing of a 90 wt% Particle Loading Nanothermite,” Advanced Materials 31, no. 23 (2019): Article 1806575, accessed 13 December 2022, https://onlinelibrary.wiley.com/doi/abs/10.1002/adma.201806575.
- Dilip Sundaram, Vigor Yang, and Richard A. Yetter, “Metal-Based Nanoenergetic Materials: Synthesis, Properties, and Applications,” Progress in Energy and Combustion Science 61 (2017): 293–365, accessed 13 December 2022, https://www.sciencedirect.com/science/article/abs/pii/S036012851530040X.
- Ibid.
- Timothy Campbell et al., “Dynamics of Oxidation of Aluminum Nanoclusters Using Variable Charge Molecular Dynamics Simulations on Parallel Computers,” Physical Review Letters 82, no. 24 (1999): 4866–9, accessed 13 December 2022, https://journals.aps.org/prl/abstract/10.1103/PhysRevLett.82.4866.
- Djalal Trache and Luigi DeLuca, “Nanoenergetic Materials: Preparation, Properties and Applications,” Nanomaterials 10, no. 12 (2020): 2347, https://doi.org/10.3390/nano10122347; Mkhitar A. Hobosyan and Karen S. Maritorsyan, “Novel Nanoenergetic Materials: Emerging Trends and Applications,” IEEE Nanotechnology Magazine 14, no. 1 (2020): 30–36, accessed 13 December 2022, https://ieeexplore.ieee.org/document/8926362.
James J. Valdes received a PhD in neuroscience from Texas Christian University and was a postdoctoral fellow at the Johns Hopkins University. He was the Army’s scientific advisor for biotechnology, an senior professional position within the Senior Executive Service, and received presidential rank awards from President George W. Bush for his development of biosensors and from President Barack H. Obama for his development and deployment to Iraq of a tactical energy system. Valdes was a participant in studies by the DOD Office of Net Assessment and, separately, the National Research Council on military applications of biotechnology, which focused on nontraditional biological research including energetic materials, biomanufacturing, smart materials, and signature management. As a senior research fellow at the National Defense University (NDU), he was senior editor of Bio-Inspired Innovation and National Security. Valdes is the author of more than 130 scientific papers, forty Army and NDU technical reports, and seven patents.
Richard S. Potember received his PhD from the Johns Hopkins University in solid-state organic chemistry. He is currently a principal engineer at the MITRE Corporation. Prior to this position, he was a program manager for solid rocket propellants and energetics in the Tactical Technology Office at the Defense Advanced Research Projects Agency. Before that, he served as a program manager and principal investigator at the Johns Hopkins applied physics laboratory. He has published more than ninety-five significant peer-reviewed in open literature (journal articles, reports, and book chapters), and holds fourteen U.S. patents. He currently teaches several graduate level courses at Johns Hopkins University in biomedical engineering and material science.
Diane M. Kotras is an intelligence strategist and policy principal with the MITRE Corporation. She specializes in AI national security policy, AI adoption, and emerging threat technologies. Her portfolio focuses on enabling AI adoption across organizations, advancements in AI technology, international AI engagement, and counterthreat. She served in the Office of the Undersecretary of Defense for Policy and the Intelligence Community prior to joining MITRE in 2017. She holds a BA degree in natural sciences from Johns Hopkins University and an MS in national security studies from the National War College.
Back to Top